Cataclysmic Variables Section
Section Leaders:
Shawn Dvorak contact
Patrick Schmeer
Interested in joining this section? Click here for instructions.
Watch the Cataclysmic Variables Section webinar (recorded September 12, 2020).
What is an accreting stellar remnant?
White dwarfs, neutron stars, and black holes are all called stellar remnants (i.e., they are considered "dead" stars) by astronomers. When these stars are isolated, their afterlives are much more sedate than if they are sharing an orbit with a nearby star (i.e, both stars are part of a binary stellar system). In this latter case, material can be transferred from the nearby star (often called a donor star), in a process called accretion. When this occurs the accreting stellar remnant can become a very interesting variable source that you can observe (the dead star becomes "alive" again). In fact, there are so many interesting accreting stellar remnants that civilian and professional astronomers alike can have difficulty navigating the "zoo" of jargon for these stellar undead. This document is a guide to help you navigate some of that jargon.
Why do regular stars "die" and form stellar remnants?
During the lifetime of most stars, the inward force of gravity is predominantly balanced by radiation forces that arise from nuclear fusion. Changes in the evolution of stars are dominated by when different parts of stars run out of fuel (at the right conditions) for these nuclear reactions. When the entire star runs out of fuel, they "die" and the star collapses due to gravity.
What are the types of stellar remnants?
The collapse of the most massive stars (stars that begin their life above about 25 times the mass of the Sun) cannot be stopped after they have run out of fuel, and they (generally) form black holes, essentially shrinking all the way down to a (zero-sized) point (or to the extremely small size — 10-35 m — where the unknown properties of quantum gravity take over). Due to mass loss in both the life and collapse of these massive stars the black holes formed this way will be between masses of about 3 and 30 times the mass of the Sun.
The collapse of the least massive stars (stars that begin their life below about 8 times the mass of the Sun) can be stopped by an effect from quantum physics (electron degeneracy pressure), such that a star the mass of the Sun collapses approximately to the size of Earth. We call the resulting objects white dwarfs . The strange properties of this quantum effect means less massive white dwarfs will have larger radii, some approaching sizes greater than that of Jupiter. The most massive white dwarfs have a mass of 1.4 times the mass of the Sun.
The collapse of the intermediately massive stars (stars that begin their life between about 8-25 times the mass of the Sun) cannot be stopped by the degeneracy pressure of electrons. But as the dead star collapses, protons and electrons can merge to form neutrons. At these intermediate masses, the degeneracy pressure from neutrons can stop collapse, but not until the dead star has become very dense. These neutron stars can have masses between about 1.4 and 3 times the mass of the Sun, but are only the sizes of cities (i.e., radii of about 10 km).
Why can the material of a nearby star accrete onto a stellar remnant?
The accreting material needs to be more gravitationally attracted to the stellar remnant than the donor star.
If the outer parts of the donor star are more gravitationally attracted to the stellar remnant, this outer material is pulled off the donor star, making a stream of material that "falls" towards the stellar remnant. This process (called Roche-lobe overflow) can occur in binary stellar systems across a wide range of separations between the two stars, depending on the properties of the donor star.
For instance, a stellar remnant can accretes from a white dwarf only if the remnant and the white dwarf are very close to one another. In this case, the separation between the system can be on the order of the distance between the Earth and the Moon, and one orbit can be completed in under about an hour! These systems are generally quite dim.
Alternatively, the stellar remnant may accrete from a normal low-mass star (i.e., a red dwarf star). Here the remnant and the star can be more widely separated, corresponding to an orbital period of a few hours to about a day.
Finally, the remnant may accrete from an low-mass star near the end of its life (i.e., a red giant star), or a very massive star, via Roche-lobe overflow at even larger separations between the two stars. In this case, the orbital period can be days to weeks.
Some high-mass donor stars in the prime of their life (blue giant star) and near the end of their life blue supergiant stars) accrete under the same Roche-lobe overflow process discussed above. However, these donor stars can have strong stellar winds, which can lead to a different type of accretion. Some portion of the donor star that has been emitted in the stellar wind can come close enough to the stellar remnant to form an accretion stream. Wind-fed accreting systems can occur when the donor star and the stellar remnant are fairly wide apart, with orbital periods of weeks to years.
Why is there so much jargon for the different types of accreting stellar remnants?
Classifying astronomical objects is an important, but difficult first step in understanding the physics behind the phenomena we observe. Some jargon was first developed to group different phenomenological behaviours together. In other words, many variable stars may have all had a similar observable property, like the shape of their lightcurves, and astronomers first grouped them together that way. Over time, we tend to regroup objects as we understand the physics behind their phenomenology. The physics (at least for major groups) can largely be described based on just a few properties. Some of the most important properties can be answered with the following:
- What is the type of stellar remnant?
- How strong is the magnetic field of the stellar remnant? How fast is the stellar remnant accreting material?
- Why is the stellar remnant accreting material (i.e., does the donor star have a strong stellar wind)?
In the sections below, this guide explains some of the jargon of the stellar undead.
Accreting White dwarfs: Cataclysmic Variables (CVs) and Symbiotic Stars (SSs)
When the accreting stellar remnant is a white dwarf, there are two major categories: Typically cataclysmic variables (often abbreviated as CVs) involve a white dwarf and a relatively low mass donor star. In this case, material from the outer envelope of the donor star can be more gravitationally attracted to the white dwarf than the donor star, leading to accretion.
The donor stars of symbiotic stars are typically more massive, and have a strong stellar wind. In this case, the wind pushes some of the stellar material from the donor star into the volume of space where the white dwarf's gravity is dominant. Symbiotic stars occur in wider binaries (i.e., larger separation between the donor star and the white dwarf) compared to cataclysmic variables.
The optical light that observers see in most accreting white dwarfs is a combination of the light from the white dwarf, the light from the donor star, and the light from the accreting material. The light from the accreting material can dominate the observed light at some times, and at other times the light from the donor star dominates the observed light. Generally the optical light from a white dwarf is the smallest component.
Since white dwarfs are both less massive and larger in radius than neutron stars and black holes, accreting white dwarfs tend to gravitationally liberate a smaller fraction of energy from the accreting material, in comparison with accreting neutron stars and accreting black holes.
If the white dwarf has a strong magnetic field (above 1 million gauss or 100 teslas — the magnetic field at the Earth's surface is about 0.5 gauss), then the material accreted from the donor star will be strongly affected. These systems are called magnetic cataclysmic variables. Close to the white dwarf, the material will flow along the magnetic field lines. And since the magnetic field structure of a white dwarf is bi-polar (i.e., there is a north pole and a south pole, just like a typical magnet), the accreted material will be funnelled to just the two magnetic poles of the white dwarf.
Polars occur when white dwarfs have the strongest magnetic fields (10-100 million gauss or 1-10 thousand teslas).
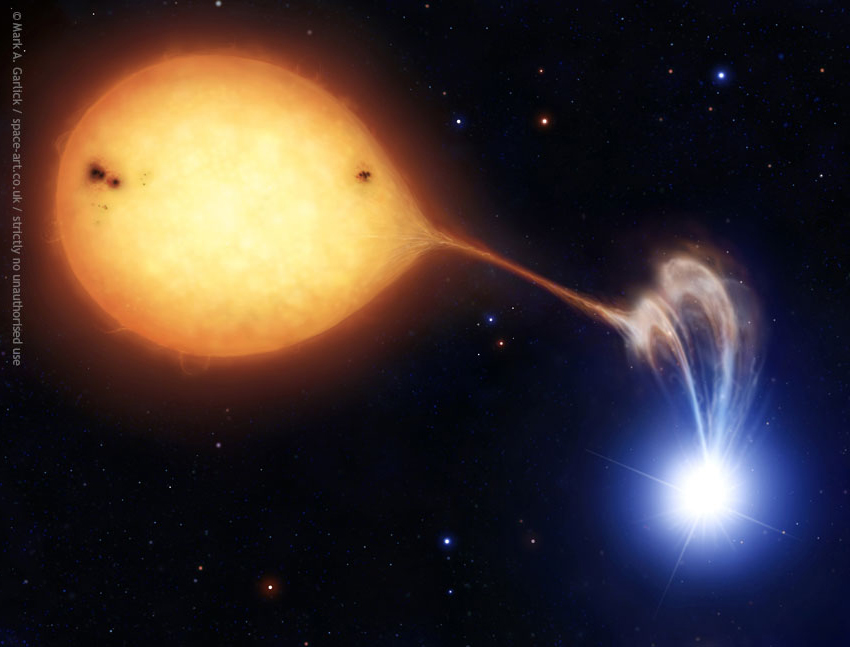
http://www.space-art.co.uk/ & http://www.markgarlick.com/
Polars occur when white dwarfs have the strongest magnetic fields (10-100 million gauss or 1-10 thousand teslas).
The exceptionally large magnetic field of the white dwarf can funnel (nearly) all of the (accretion) stream of material from the donor directly onto the two magnetic poles, without intermediate steps. The extreme magnetic-dominated accretion onto the poles of the white dwarf shocks the accreting material and generates copious optical light. AM Herculis is a prototypical example of a polar CV that has been well studied by the AAVSO.
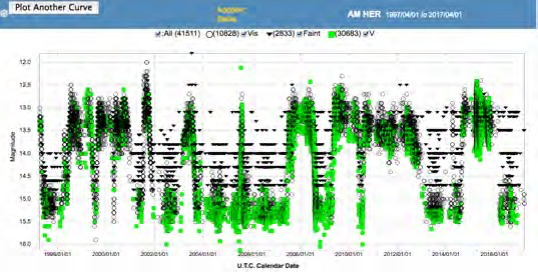
Intermediate Polars occur when white dwarfs have strong magnetic fields that are about a factor of 10 weaker than polar CVs (between about 1-10 million gauss or 1000-1000 teslas). However, the magnetic field is not strong enough to form accretion streams that go directly between the donor and the two magnetic poles on the surface of the white dwarf.
Instead, the accretion stream from the donor first settles into a flattened disk that rotates around the white dwarf. The strong magnetic fields close to the white dwarf essentially truncate the accretion disk, and the material then follows the magnetic field lines from the disk to the magnetic poles of the white dwarf. DQ Herculis is a prototypical example of an intermediate polar CV that has been well studied by the AAVSO.
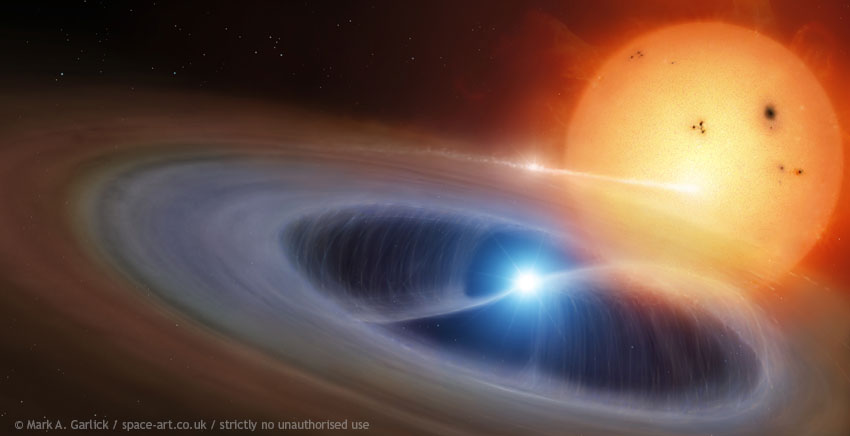
http://www.space-art.co.uk/ & http://www.markgarlick.com/
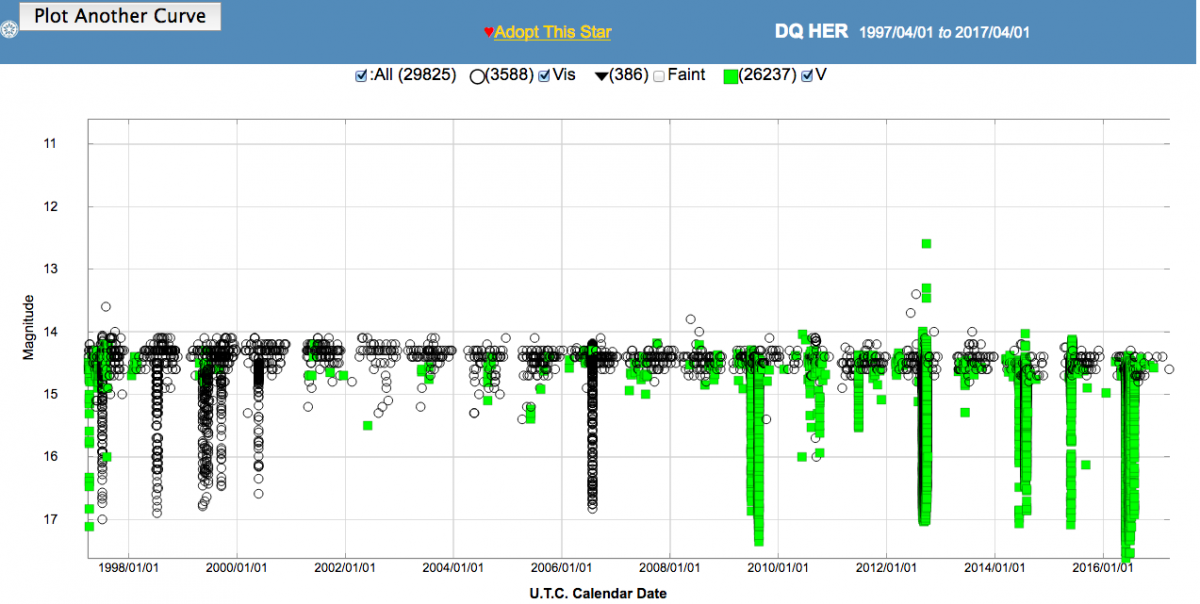
White Dwarf Pulsars are a brand new (as of 2016) class of a close binary system involving a magnetic white dwarf. In fact, the star AR Scorpii is the only known example of this type of system so far. The white dwarf is spinning relatively rapidly (once every 1.97 minutes). Once per period we see a pulse of emission from the white dwarf across many electromagnetic wavelengths. But unlike intermediate polars, this system does not seem to be accreting strongly onto the surface of the white dwarf. Citizen astronomers played a key role transforming AR Scorpii from a well-studied (mis-classified) system into the prototype for an intriguing new class of variable stars!
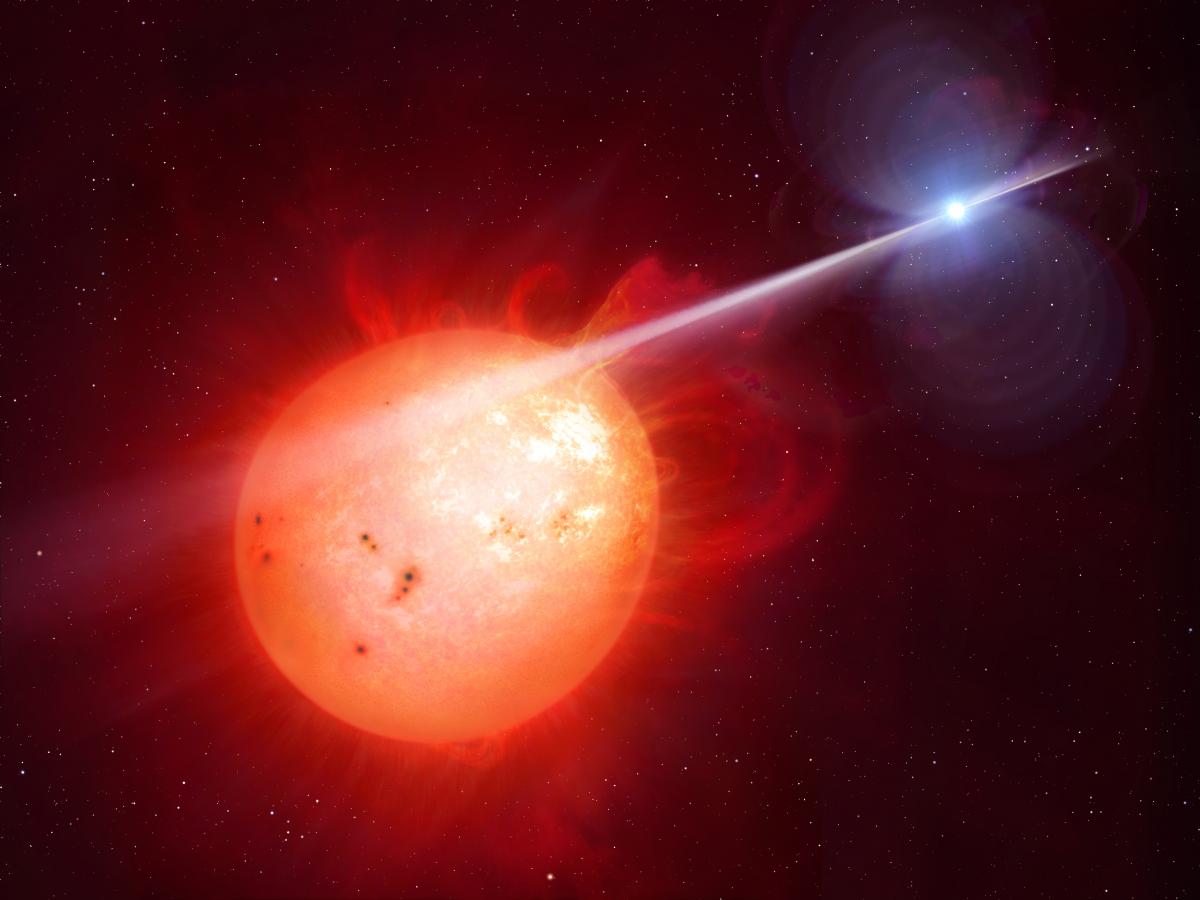
Garlick/University of Warwick/ESO
Non-Magnetic Cataclysmic Variables
If the white dwarf does not have a strong magnetic field, then the material streaming from the donor star will settled into a flattened disk that rotates around the white dwarf. Unlike intermediate polars, the inner edge of the accretion disk will generally reach the white dwarf surface. Without strong magnetic fields, non-magnetic CVs can accrete material over their entire surface. Another critical difference is that the matter falling onto the white dwarf is no longer controlled by magnetic fields. Instead, the viscosity of the accretion disk typically controls the rate at which the white dwarf accretes. In many CVs, the optical light arises from three sources: the hotspot where the accretion stream hits the accretion disk; the accretion disk itself; and the surface of the white dwarf. In some CVs, the dominant energy source is not the gravitational energy liberated by material falling onto the CV. Instead, nuclear fusion reactions can be a major source of energy.
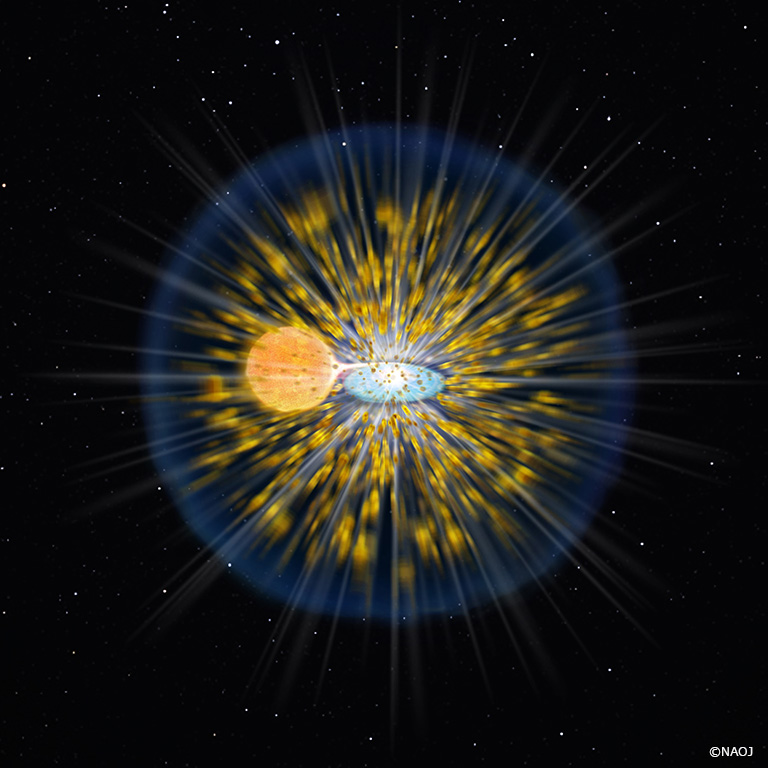
Ⓒ NAOJ
Classical Novae occur when runaway nuclear fusion (a thermonuclear explosion) occurs on the surface of the white dwarf. In these systems, the gravitational energy liberated by accreting material from the donor star heats the white dwarf. The white dwarf becomes so hot that its top layer (which will tend to be dominated by hydrogen atoms) will be able to undergo a thermonuclear explosion. The energy of this explosion will dominate over the gravitational energy. The CV will rapidly get extremely bright optically and part of the white dwarf's atmosphere will be expelled into space. Some non-magnetic CVs have undergone only one classical novae over a century of observations, while other non-magnetic CVs can undergo recurrent novae. Most CVs undergoing novae show continuous accretion through the accretion disk, and are thus relatively steady sources outside the nova eruption itself. RS Ophiuchi is a prototypical recurrent nova, having undergone about 8 classical novae since 1898.
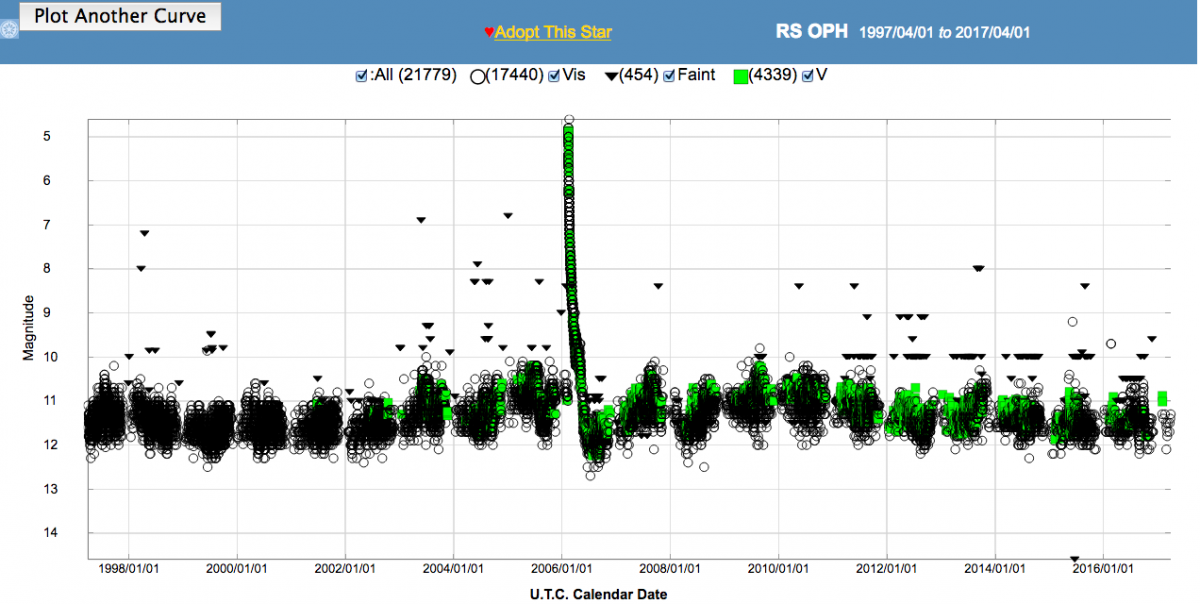
Dwarf Novae are a type of non-magnetic CV where the variability observed is due to variations in the accretion flow onto the white dwarf. For long periods of time, a dwarf nova system will only slowly accrete matter onto the white dwarf. But a change in the viscosity of the accretion disk dramatically increases the rate of mass transfer through the disk, heating the disk. This leads to strongly increased optical output that is typically not as bright as in a classical nova. These fainter outbursts are the origin of the name of this class of systems. Many dwarf nova undergo these outbursts frequently, with varying times between outbursts. SS Cygni is one of the most well-studied dwarf novae. The AAVSO has an exceptionally long history of observations on this source, and AAVSO members have helped astronomers change our understanding of this source.
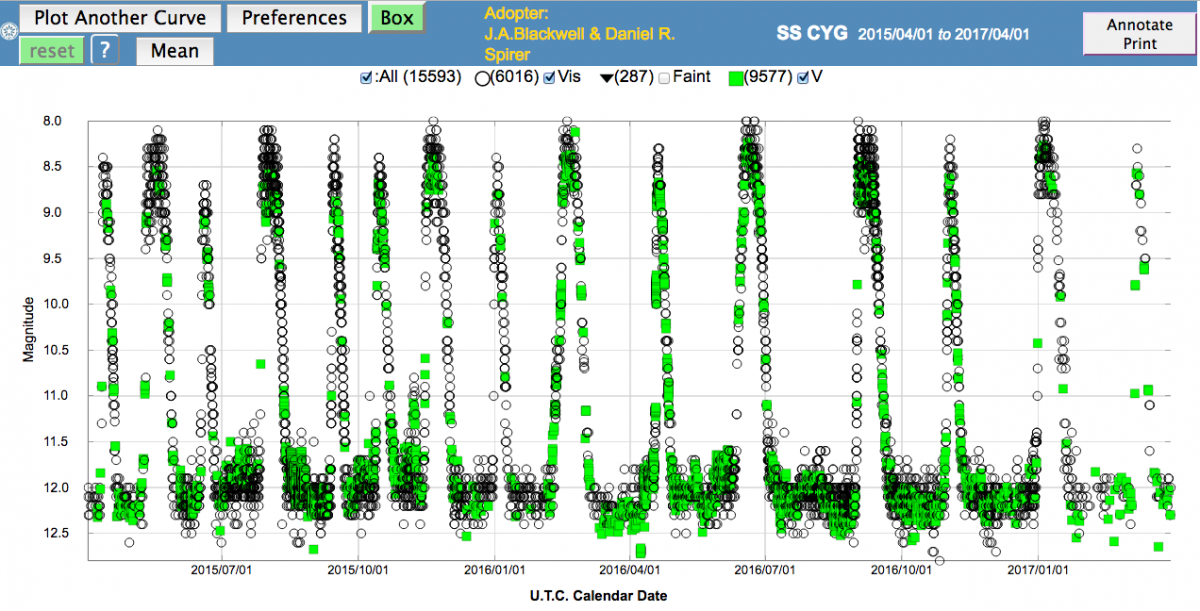
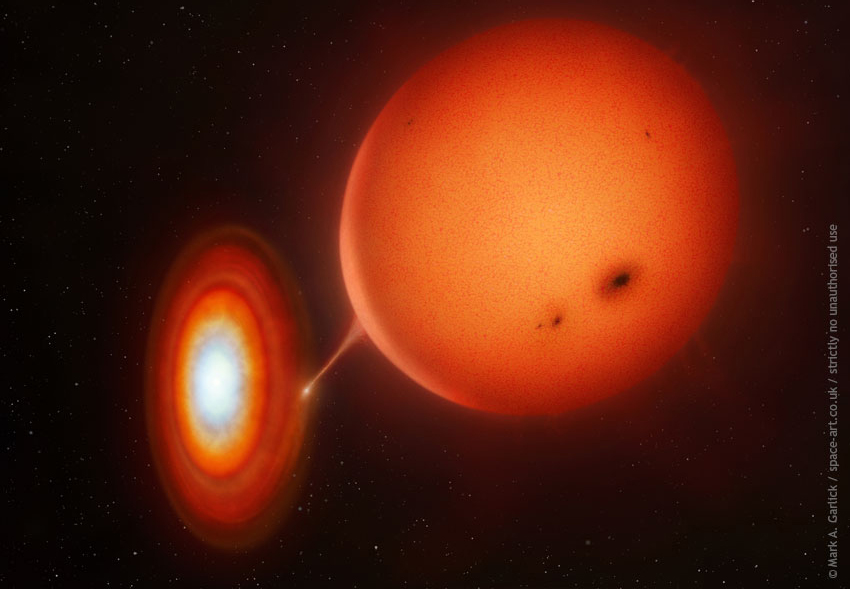
http://www.space-art.co.uk/ & http://www.markgarlick.com/
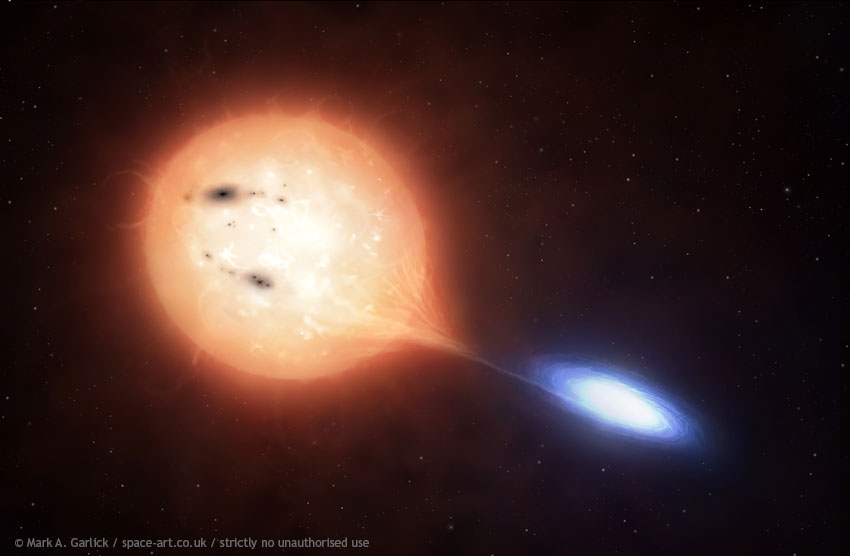
http://www.space-art.co.uk/ & http://www.markgarlick.com/
Nova-like CVs tend to have continuous mass transfer through their disks, like in CVs that have exhibited nova eruptions, but have not been seen to show a nova eruption. Essentially, nova-like CVs are cataclysmic variables that are persistently undergoing dwarf nova outbursts. RW Sextanis is a nova-like CV that has been a target of recent AAVSO campaigns.

Symbiotic Stars
Red giants, stars near the end of their lives, have much stronger stellar winds than most stars in the prime of their lives. This allows a white dwarf to accrete at high rates from the wind of the red giant. Such accreting white dwarfs can occur in binary systems where the donor star and the white dwarf are separated by a much farther distance (what is called a "wide binary"). These Symbiotic Stars, like V407 Cygni (picture below) can undergo some of the same behaviour seen in other accreting white dwarfs, like classical nova.

Credit: NASA's Goddard Space Flight Center/S. Wiessinger
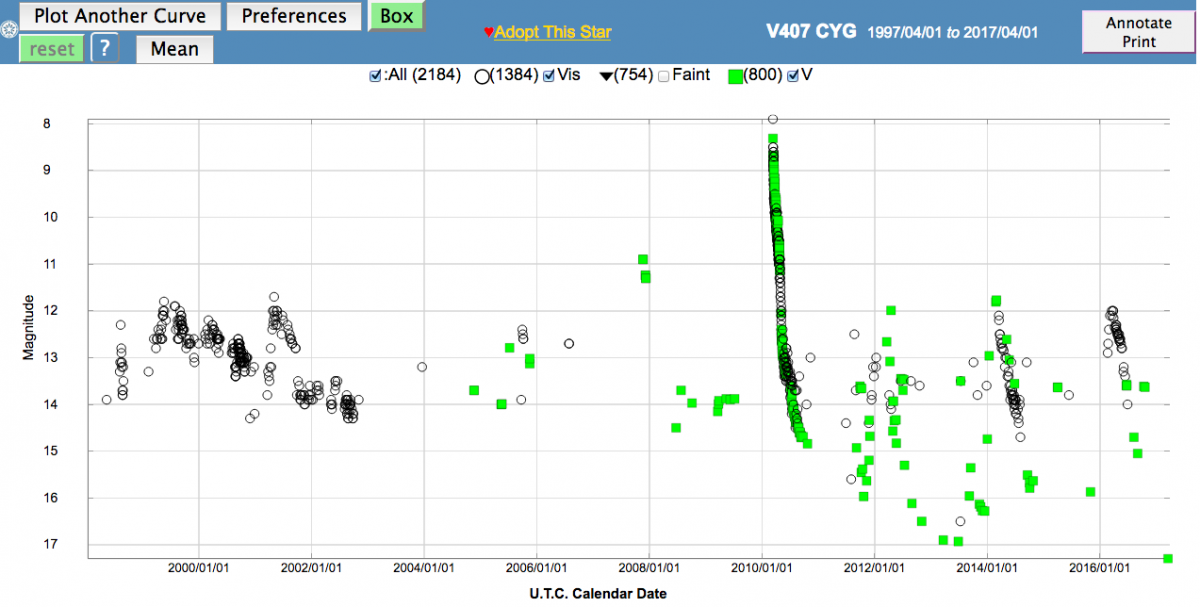
Acknowledgments:
Gregory Sivakoff acknowledges assistance provided by Craig Heinke and Stella Kafka in developing this guide. All images used in this document are either AAVSO generated, images in the public domain (e.g., through organizations like NASA and NAOJ), or have explicit permission for use from the illustrator.
Dr. Mark A.Garlick http://www.space-art.co.uk/ & http://www.markgarlick.com/